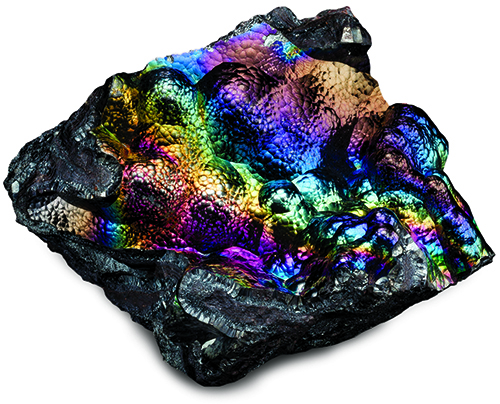
Iridescent minerals fit the familiar expression “beauty is in the eye of the beholder.” It is one of the mineral kingdom’s most fascinating displays of color. The glow, shimmer, sheen, aura and glitter of iridescence has been compared to everything from rainbows, fire, neon lights, and pyrotechnics to kaleidoscopic displays of the northern lights.
The word “iridescence” stems from the Latin words iris, or “rainbow,” and descendere, or “coming down.” Its literal meaning, “from the rainbow,” fits an optical phenomenon that manifests itself as a rainbow-like play of vivid colors.
Nature of Iridescence
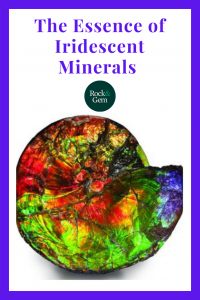
While iridescence occurs throughout the natural world, notably in certain bird feathers, flower petals, reptile scales, and seashells, it is particularly apparent in minerals. Iridescence can be a diagnostic property in chalcopyrite, bornite, covellite, and other metal sulfides. It can increase the value of mineral specimens and is the basis of the visual appeal of such gem materials as opal, labradorite, and fossil “ammolite.”
Iridescence differs from other optical phenomena in the nature of its colors. Most colors are spectral or pigmentary in origin and are produced, respectively, by the diffraction and absorption of white light. But iridescent colors, also called structural colors, have a structural origin and are created by reinforcing reflected light waves.
Light and Iridescence
Light is a form of electromagnetic radiation that is detectable by the human eye. Light exhibits properties of both waves and particles and travels in waves that are measured by frequency (wavelengths), phase (positions of wave crests and troughs), and amplitude (wave magnitude).
The wavelengths of visible light extend from 380 nanometers (red) to 760 nanometers (violet). “White” light, a mix of all visible wavelengths, can be diffracted or separated into its spectral color components of red, orange, yellow, green, blue, indigo, and violet.
Examples of Spectral Colors
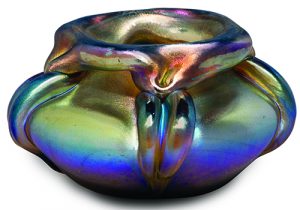
Examples of spectral colors are the hues of rainbows and the spectrum created when a prism diffracts light. Pigmentary colors are produced when materials absorb specific wavelengths of white light and reflect others. Objects that we perceive as red absorb the orange, yellow, green, blue, indigo, and violet wavelengths and reflect only the red wavelengths.
Structural colors have an entirely different origin and are created when certain microscopic structures modify light waves, reflecting and becoming reinforced.
Peacock feathers are textbook examples of how pigmentary colors and structural colors differ. The pigmentary color of peacock feathers is dull brown, but the interaction of light with microstructures on the feather surfaces creates the intense, green-and-blue structural colors of iridescence.
Understanding Iridescence
The cause of iridescence was a scientific puzzle for centuries. In 1665, English naturalist Robert Hooke noted that the “fantastical” iridescent colors of peacock feathers were entirely unrelated to their brown pigmentary color. A half-century later, English mathematician and physicist Sir Isaac Newton, a proponent of the particle theory of light, refined Hooke’s idea by concluding that peacock-feather surfaces somehow modified light to cause iridescence.
In 1804, English physicist Thomas Young, who believed that light behaved as a wave, observed that peacock-feather surfaces consisted of repetitive, microscopic ridges that diffracted light into its spectral components. After reflecting, these components seemed to interact to gain brilliance and intensity. In 1892, English zoologist Frank Evers Beddard coined the term “structural colors” to describe iridescence’s vivid color.
Optical Interference and Iridescence
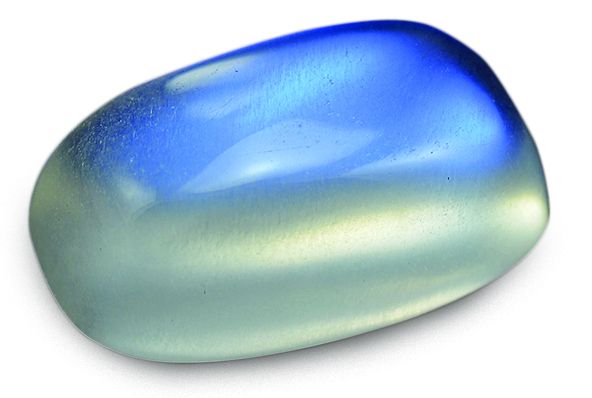
By 1900, scientists understood that iridescent structural colors resulted from the phenomenon of optical interference. Optical interference occurs when two or more reflected light waves of the same frequency and traveling in parallel paths overlap in coordinated phases.
Optical interference can be destructive or constructive. In destructive interference, out-of-phase wavelengths cancel each other out. In constructive interference, the cause of iridescence, the overlapping waves are in phase and reinforce each other.
Reinforcement increases wave amplitude to create structural purple, cyan, green, metallic copper and gold, and magenta colors. Because constructive interference reinforces only a single wavelength at a time, the resulting structural color has greater vibrancy than the corresponding spectral or pigmentary colors and quality described as “pure,” “electric,” “vibrant,” and “neon-like.”
Thin-Film Interference
Two different types of microscopic structures produce optical interference: thin films and diffraction gratings. In thin-film interference, light strikes a surface consisting of two superposed, parallel, reflective planes separated by a distance roughly equal to the wavelengths of light.
The upper layer reflects some of the incident light and transmits the remainder to the underlying layer. These planes then reflect two slightly offset beams traveling in the same direction. Reinforcement occurs when specific wavelengths within these beams are in a coordinated phase.
Diffraction-Grating Interference
Unlike thin-film interference, diffraction-grating interference requires a single, reflective surface composed of periodic, parallel, nanoscale ridges or grooves separated in distance by the wavelengths of light.
The edges of these ridges diffract light into its wavelength components; reinforcement occurs when the reflected beams are in a coordinated phase. Despite their different structural origins, the iridescence produced by both thin-film and diffraction-grating interference is identical.
Iridescent Sulfide Minerals
Iridescence can appear in a surprisingly large number of minerals with differing chemistries, crystal structures, transparency and luster.
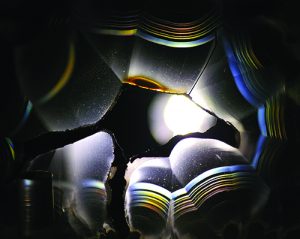
It is most common in opaque metal sulfides with a metallic luster. These include chalcopyrite [copper iron sulfide, CuFeS2], bornite [copper iron sulfide, Cu5FeS4], covellite [copper sulfide, CuS], stibnite [antimony sulfide, Sb2S3], bismuthinite [bismuth sulfide, Bi2S3], pyrite [iron disulfide, FeS2], and the skudderite series of cobalt-nickel arsenides.
The surfaces oxidize or tarnish into microscopically thin films of metal oxides. In chalcopyrite, surface oxidation creates a film of hematite [iron oxide, Fe2O3], goethite [basic iron oxide, FeO(OH)], and limonite (a mix of ferric and ferrous oxides and hydroxides).
Repetitive oxidation phases and subsequent weathering can create multiple, superposed oxide layers. When properly spaced, these layers cause thin-film interference.
Not Always Iridescent
Chalcopyrite and other metal sulfides are not always iridescent. Iridescence occurs only in specimens in which the distance between the surface oxide films approximates that of the wavelengths of light. Variations within this distance determine the intensity and specific wavelengths of the resulting structural colors.
Rotating a specimen of chalcopyrite changes the incident-light angle and thus the distance that light travels between the two reflective surfaces. This movement varies the frequencies of the reinforced wavelengths to create kaleidoscopic displays of continuously changing colors.
Structural Color Ranges
Many metal sulfides tend to exhibit specific structural-color ranges. Chalcopyrite’s iridescent colors usually consist of gold and magenta, while bornite’s lean toward gold and cyan with only occasional flashes of magenta.
In covellite and stibnite, the distancing of the respective copper-oxide and tin-oxide layers produces a cyan or purple iridescence. The layer separation in polybasite (a complex silver copper antimony arsenic sulfide) creates a predominantly green iridescence.
Surface & Internal Iridescence
While opaque metal sulfides show only surface iridescence, translucent or transparent minerals can display surface and internal iridescence. Among these is the labradorite variety of the feldspar-mineral anorthite [calcium aluminum silicate, CaAl2Si2O8].
The internal layers of labradorite are twinned, translucent microcrystals create both thin-film and diffraction-grating interference and a distinctive type of iridescence called labradorescence, a multicolored, subsurface sheen that sweeps broadly across the entire stone. Most labradorite exhibits cyan, green, and gold structural colors with only hints of magenta. The intensity of labradorescence varies greatly among individual specimens.
Labradorite’s History
Labradorite’s first reference is on the Isle of Paul in today’s Canadian province of Newfoundland and Labrador. The Isle of Paul’s granite bedrock consists mostly of anorthite, which, at certain angles of sunlight, glows with labradorescence.
The native Inuit people likened its eerily shifting, structural colors to those of the northern lights. Cut-and-polished labradorite often appears in pendants. Specimens with particularly intense labradorescence are known in the jewelry trade as “spectrolite.” The national gemstone of Finland is a type of spectrolite that is unusually rich in gold and magenta.
Several commercial architectural stones, such as “blue granite” and “labradorite granite,” contain evenly dispersed labradorite phenocrysts that create a subtle but attractive labradorescence.
This story about iridescent minerals is part of a two-part series that previously appeared in Rock & Gem magazine. Click here to subscribe. Story by Steve Voynick.